Research - Neutrinos
Since the postulation of neutrinos in 1930 by Pauli the neutrino has been at the center of the investigation in many particle and astroparticle physics experiments. So far, neutrinos are best described by the standard model of particles, where they are specified as charge neutral, massless fermions, which are created by weak interaction in one of the three different leptonic eigenstates. However, the experiments of the Sudbury Neutrino Observatory and Super-Kamiokande showed that the leptonic neutrino flavor changes during propagation. This effect indicates that neutrinos have at least three additional mass eigenstates with non-zero mass-squared differences. Thus, the experiments proved that neutrinos have non-zero masses, contradicting the standard model. Until now, the absolute mass is still undetermined, and searched for in various experiments.
Neutrino mass experiments can be classified into two conceptually different categories: indirect and direct approaches. In the indirect search, the neutrino mass is inferred from a model, where the model itself is not fully proven. The indirect approaches include cosmological studies, supernova neutrino measurements and measurements of the neutrinoless double beta decay. In the direct search, the neutrino mass is determined directly from well tested models. The direct approaches includes the spectroscopy of beta decay electrons.
Beta decay experiments investigate the missing energy, which will be carried away by the neutrino, in either the electron capture process or the beta decay process. The calculations are based on energy and momentum conservation. No assumption is made on the specific nature of neutrinos. Thus, these methods can be considered as model independent. In the following, only the beta decay measurement principle will be described because both methods are so fundamentally similar.
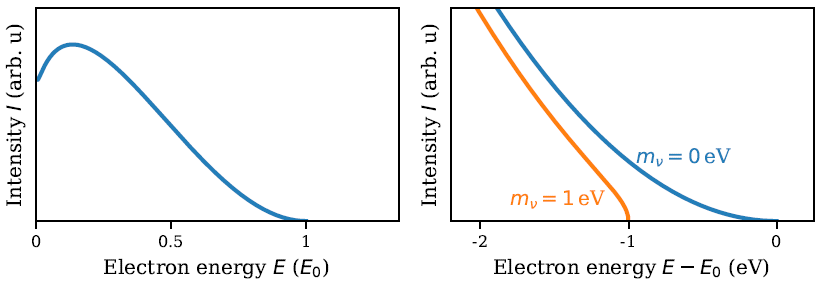
The distortion as well as the endpoint shift can be detected, given a high enough event rate in the endpoint region as well as a high enough energy resolution of the experiment. Thus, the choice of beta decaying isotopes relies on a low half-life and a low endpoint energy. The low half-life guarantees that there is enough statistics in the endpoint region with a comparably small amount of source material. The low endpoint energy ensures that the influence on the spectrum is comparably large.
Tritium is an ideal candidate for neutrino mass measurements. It has a short half-life of T1/2= 12.3 years and has a low endpoint energy of E0= 18.6 keV. Because of these properties, tritium is used in many beta decay experiments and most recently at the KATRIN experiment. The KATRIN collaboration could show that the electron antineutrino mass is below 1.1 eV (90\% confidence level). However, the KATRIN experiment aims to measure the electron antineutrino mass with a sensitivity down to 0.2 eV. This challenging goal can only be reached through a precise examination of all systematic effects of the experiment. One of these effects is caused by a plasma in the high luminous windowless gaseous tritium source. The plasma is generated by beta decay, subsequent ionization and collisions with surrounding tritium gas. The plasma produces an ab initio inhomogeneous potential throughout the source, which can change the shape of the measured electron spectrum.
The plasma inside the WGTS source of the KATRIN experiment is very intricate in many aspects
- The plasma has a very strong density gradient
- The plasma is collisional in the center and collisionless at the edges
- The electron distribution has a Maxwellian part and several nonthermal components
- A large number of different species is present
- The interaction of the plasma with the walls
In my group a two-part simulation approach was developed. First, the electron spectrum is reevaluated, dependent on the position in the source by a new Monte-Carlo simulation tool, named KARL. It will cover the interactions of electrons and ions with the neutral gas by a given neutral gas flow and static electromagnetic fields. Second, the results of KARL are then incorporated in particle-in-cell simulations with a modified version of the ACRONYM simulation tool. This tool will calculate electrodynamic fields from given input, assuming that there are no direct collisions between charged particles. Results of the ACRONYM simulations can then be used as an input for new KARL simulations. It is hypothesized that the iteration between both simulations tools will reach an equilibrium state.
The KARL code includes various processes from ionization, recombination and elastic scattering. In order to measure density and spectrum at different positions in the source perticles are tracked at different checkpoints.
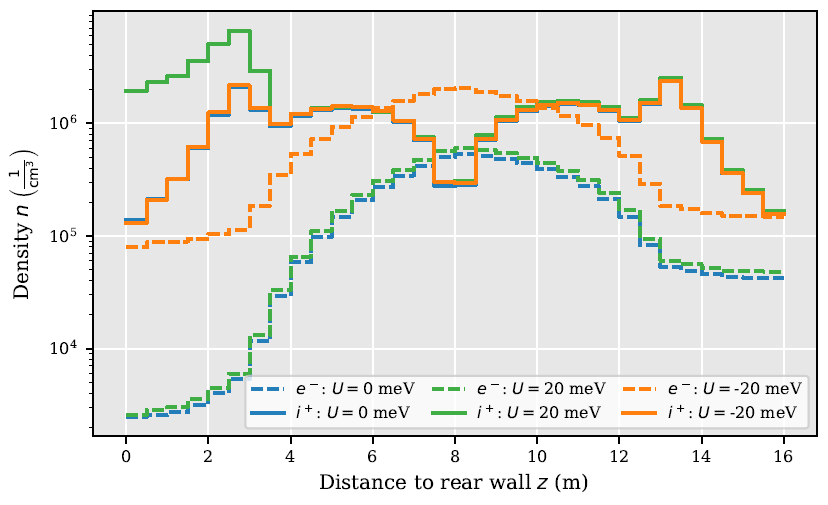
The spectrum inside the source shows a complex behaviour: Electrons are emitted according to the Fermi spectrum. Through interactions with the ambient tritium gas and ions the electrons lose energy leading to a nonthermal power-law. After a large number of elastic scattering events - mainly in the center where the gas density is high - electrons cool down to a Maxwell distribution.
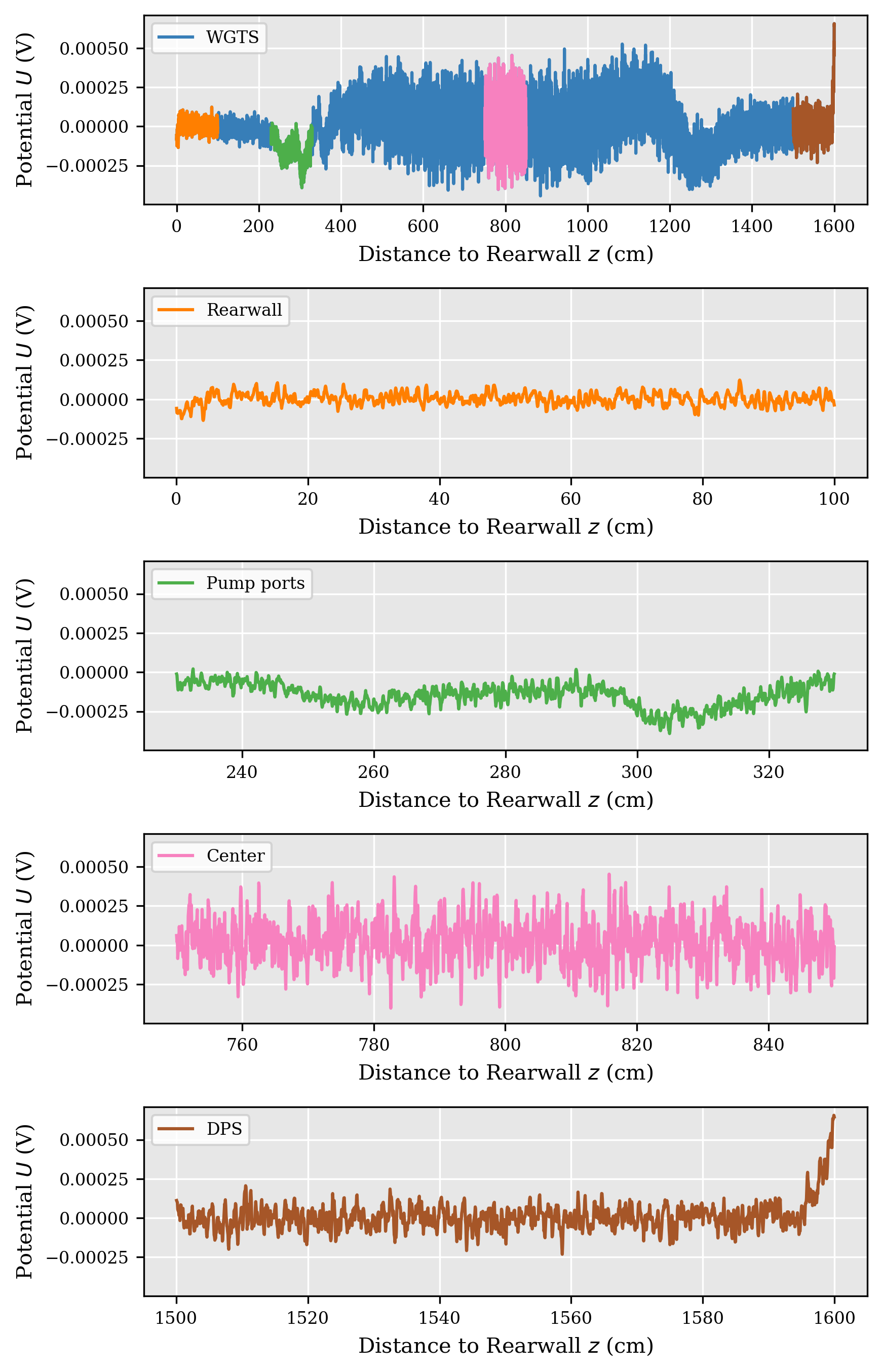
Particle spectra are used as input for plasma simulations with the ACRONYM code. Using appropriate boundary conditions the behaviour on large scales can be simulated. With the current setup numerical experiments are computationally expensive. We are currently working on making simulations more feasible using various approaches from pseudo-2D PiC simulations to 1D electrostatic Vlasov simulations.